Chemical Imaging of Spatial Heterogeneities in Catalytic Sol
更新时间:2023-04-06 21:00:01 阅读量: 教育文库 文档下载
- chemical推荐度:
- 相关推荐
Heterogeneous Catalysts DOI:10.1002/anie.200900339Chemical Imaging of Spatial Heterogeneities in Catalytic Solids at Different Length and Time Scales
Bert M.Weckhuysen*
Angewandte
Chemie Keywords:
heterogeneous catalysis ·
in situ spectroscopy ·
nanoparticles ·
single-molecule
spectroscopy ·
zeolites
B.M.Weckhuysen Reviews
d5bbf012b90d6c85ec3ac6cb 2009Wiley-VCH Verlag GmbH &Co.KGaA,Weinheim Angew.Chem.Int.Ed.2009,48,4910–4943
1.Introduction 1.1.Spatial Heterogeneities in Catalytic Solids
Heterogeneous catalysts are the workhorses of chemical
industry,and more than 80%of all chemicals have come into contact with at least one catalyst material during their manufacturing process.[1–6]Catalytic solids are very complex,as they possess many different potential active sites in their porous structures.[7]Determining the nature of these active sites and elucidating their reaction mechanisms are important intellectual challenges that are of paramount importance for the rational design of heterogeneous catalysts.Success in these endeavors offers the rewarding prospect of improved formulations of existing catalytic solids and the possibility to create more effective and selective catalysts from scratch.Until recently,most characterization studies of catalytic solids focused on ensemble-averaged measurements,assum-ing that heterogeneous catalysts are spatially homogeneous objects when placed in a catalytic reactor.Point measure-ments (0D scans)of catalytic solids are then usually taken as a function of reaction time to study activation and deactivation phenomena.Such measurements allow the development of structure–performance relationships by relating different physicochemical properties to each other,such as activity,
selectivity,porosity,adsorption,degree of reduction,and a
multitude of spectroscopic signatures.[8–11]However,these
macroscopically determined properties are not necessarily identical across the entire reactor bed or within a catalyst grain.Spatiotemporal phenomena are more the rule than the exception for heterogeneous catalysts,as the local environ-ment strongly affects the above-mentioned macroscopic properties.All this leads to spatial heterogeneities or reaction fronts,which remain unnoticed when point measurements are performed.
An archetypal example of spatiotemporal variations in heterogeneous catalysis is the catalytic oxidation of CO with O 2over platinum model catalysts as investigated by Ertl and co-workers.[12]Figure 1shows a 2D photoemission electron microscopy (PEEM)image of the formation of spiral waves in catalytic CO oxidation on a Pt(110)surface.Dark areas are essentially oxygen-covered,while the lighter patches are CO-covered.The spiral waves develop and propagate with front speeds of a few micrometers per second,and the cores of the
spirals are often formed at a region on the Pt surface with enhanced defect density.This effect is also responsible for the fact that the wavelengths of the spiral waves vary to some degree.As the reaction mechanism of CO oxidation is known in great detail and all its parameters of influence have been determined,it has been possible to simulate the process of spiral wave development,which nicely matches the exper-imental findings.
K nowledge of spatiotemporal gradients in heterogeneous catalysts is of paramount importance for the rational design
of new and more sustainable catalytic processes.Hetero-geneities resulting in space-and time-dependent phenomena occur at different length scales;that is,at the level of catalytic reactors (mm to m),catalyst bodies (m m to mm),catalyst
grains (nm to m m),and active sites and metal (oxide)particles ( to nm).This Review documents the recent advances in the development of space-and time-resolved spectroscopic methods for imaging spatial heterogeneities within catalytic processes at these four length scales.Particular emphasis will be on the use of magnetic resonance,optical,and synchro-tron-based methods,their capabilities in providing spatial resolution (1D and 2D imaging)and depth profiling (3D imaging)as well as on their time-resolved application,potential for single-molecule and nanoparticle detection,and use under reaction conditions.The Review ends with future prospects on spectroscopic markers for catalytic activity,
label-free spectroscopy,tomography at the nanoscale,and correlative microscopic approaches.From the Contents
1.Introduction 49111.1.Spatial Heterogeneities in Catalytic Solids 4911
1.2.Scope 4913
2.Imaging Spatial Heterogeneities of Catalytic Solids at Different Length and Time Scales with Electromagnetic
Radiation 4913
2.1.Spatial Heterogeneities at the Level of a
Catalytic Reactor 49142.2.Spatial Heterogeneities at the Level of Millimeter-Sized Catalyst Bodies 49212.3.Spatial Heterogeneities at the Level of
Micrometer-Sized Zeolite Crystals 4925
2.4.Spatial Heterogeneities at the Level of Catalyst Nanoparticles and Single Molecules 4932
3.Future Prospects 4937
3.1.Microscopy of Labeled Samples and
Label-Free Microscopy 4937
3.2.Towards In Situ Optical Tomography at
the Nanoscale 4938
3.3.Development of Correlative Microscopy
Approaches 4939
[*]Prof.Dr.B.M.Weckhuysen
Inorganic Chemistry and Catalysis group
Debye Institute for Nanomaterials Science,Utrecht University Sorbonnelaan 16,3584CA Utrecht (The Netherlands)
E-mail:
b.m.weckhuysen@uu.nl
Supporting information for this article is available on the WWW under d5bbf012b90d6c85ec3ac6cb/10.1002/anie.200900339.
Spatial Heterogeneities in Catalysis Angewandte Chemie 4911Angew.Chem.Int.Ed.2009,48,4910–4943 2009Wiley-VCH Verlag GmbH &Co.KGaA,
Weinheim
Spatiotemporal effects are,however,not limited to model surface-science studies,but are generally expected for open systems far from equilibrium,as is the case for all heteroge-neous catalytic processes.In realistic heterogeneous catalytic systems they occur at four different length scales,as schemati-cally illustrated in Figure 2.To better visualize these different length scales,Movie S1is available as Supporting Informa-tion,showing how a propane molecule travels through an industrial dehydrogenation plant until it is finally converted into propene and hydrogen.[13]The first level of interest is the reactor,in which a suitable catalyst material is carefully packed.A catalytic reactor is usually on the order of centimeters to meters in length if a full-scale commercial installation is considered.The catalyst performance,however,is not the same over the entire catalyst-bed volume.When considering a fixed-bed reactor,as is the case for the propane
dehydrogenation plant,spatiotemporal gradients in the con-centrations of propane,propene,hydrogen,and by-products (e.g.ethane and methane)are observed.In other words,the concentrations of these molecules vary along both the axial and radial directions of the catalyst bed as a function of reaction time.Moreover,temperature gradients may also arise from the endothermicity of the dehydrogenation process.As a result,catalyst reduction and deactivation phenomena may be quite different along the catalyst bed,significantly affecting catalyst regeneration procedures.
To avoid pressure drops in gas-phase reactors,catalyst bodies of millimeter dimensions are used.They often have particular shapes,and reactant molecules must penetrate through these catalyst bodies to be converted.In some cases,the catalytically active phase has been specifically positioned on the outer layers of the catalyst body,leading to a particular spatial distribution of the active phase (i.e.an egg-shell distribution).The third level at which spatial heterogeneities may occur constitutes,for example,porous catalyst crystals or grains of micrometer or sub-micrometer dimensions.
Diffu-Figure 1.2D Photoemission electron microscopy (PEEM)image from a Pt(110)surface during catalytic CO oxidation (p CO =4.3 10à4,
p O 2=4 10à5mbar,T =1758C).The diameter of the picture is 500m m.Reproduced from reference
[12].
Figure 2.Selected examples of spatial heterogeneities occurring in heterogeneous catalysis at different length scales:a)Selected frames from Movie S1in the Supporting Information showing how a propane molecule travels through an industrial dehydrogenation plant until it is finally converted into propene and hydrogen.b)Coke and temperature profiles over an industrial reactor spanning several meters.c)Spatial gradients of the amount of transition-metal ion across an inpidual Al 2O 3catalyst body spanning several millimeters.d)Spatial gradients of a reaction product across an inpidual zeolite crystal spanning several micrometers.e)Spatial gradients of metal,metal oxide,and metal carbide within an inpidual catalyst grain spanning several
nanometers.
Bert Weckhuysen,born in 1968in Aarschot,Belgium,received his master degree from Leuven University (Belgium)in 1991.After finishing his PhD studies under the super-vision of Prof.Schoonheydt in 1995,he worked as a postdoctoral fellow with Prof.Wachs at Lehigh University (USA)and with Prof.Lunsford at Texas A &M University (USA).From 1995until 2000he was a research fellow of the Belgian National Science Foundation.Since 2000,Weckhuy-sen has been full professor inorganic chemis-try and catalysis at Utrecht University.He
received several research awards,including the 2002VICI award from the Netherlands Organization for Scientific Research (NWO),the 2006Gold Medal from the Royal Netherlands Society of Chemistry (KNCV),the 2007DECHEMA Award from The Max Buchner Research Foundation (Germany),and the 2009Netherlands Catalysis and Chemistry Award from the KNCV Catalysis Section and the Netherlands Organization of Catalysis Industries.He is scientific director of the Dutch Research School for Catalysis (NIOK)and an elected member of the Young Academy of the Royal Dutch Academy of Sciences (KNAW),the Netherlands Academy of Technology and Innovation (NATI),and the Royal Holland Society of Sciences (KHMW).
B.M.Weckhuysen
Reviews
d5bbf012b90d6c85ec3ac6cb
2009Wiley-VCH Verlag GmbH &Co.KGaA,Weinheim
Angew.Chem.Int.Ed.2009,48,4910–4943
sion of reactants and reaction products within these porous networks,which contain a wide distribution of macro-,meso-, and micropores,will strongly influence the overall perfor-mance of a catalytic process.Short-and long-range cracks, transport barriers,and dead ends in the pore structure may lead to different diffusion pathways and related concentration gradients,directly influencing catalyst performance and even making some areas of the catalyst grain completely inacces-sible for catalytic action.In other words,catalyst bodies and grains may be inefficiently used when transport phenomena become rate-limiting.
Finally,the catalyst grains contain active sites with dimensions of nanometers or ngstr?ms.This is the fourth important length scale at which spatial heterogeneities may occur.Although the active site can be spatially isolated,such as for Br?nsted acid groups in zeolites,in most cases the activity of this site is still distinct from another site spatially positioned only a little further in the catalyst grain.This phenomenon can be due to local changes in chemical composition or to different reactant accessibility induced by shape-selectivity effects.Furthermore,the number of active sites can differ from surface to surface.In the case of supported metal nanoparticles,for example,the reactivity and selectivity can be tailored by controlling the shape,as shape determines the number of atoms located at the edges and corners.[14]In general,high-index planes have a greater density of unsaturated atomic steps,edges,and kinks,which can serve as active sites for the breaking and making of chemical bonds.[15]Furthermore,these supported metal nano-particles may coexist in a wide variety of sizes and shapes, each possessing its own catalytic activity.The same hetero-geneity may result when an alloying element is introduced to the metal nanoparticle,as the alloy can be uniform or have, for example,an egg-shell distribution.
1.2.Scope
It is no wonder that the complexity described above complicates the development of useful structure–perfor-mance relationships in the field of heterogeneous catalysis, as the measured macroscopic properties,such as activity and selectivity,are the result of a complex interplay between different physicochemical gradients across the four important length scales.Therefore,mastering the overall performance of a catalytic process in terms of activity,selectivity,and stability requires full control of the spatiotemporal phenomena taking place from the meter scale down to the level of a few nanometers or even smaller.Evidently,this is an enormous task,but fortunately both academic and industrial researchers have built up a lot of(often empirical)knowledge and technical skill to tackle the related challenges to construct robust and efficient catalytic processes.
This Review will show the tremendous progress we have seen in the last decade in the field of spectroscopy for imaging spatial heterogeneities in catalytic solids,preferably applied under in situ conditions,leading to new physicochemical insights at the four length scales of importance in heteroge-neous catalysis.This will be done by highlighting space-and time-resolved spectroscopy research on molecular transport, catalyst preparation,and reactivity and deactivation phenom-ena.Special emphasis will be placed on combining micro-scopy and spectroscopy,including1D(x scanning),2D(x-y scanning),3D(x-y-z scanning or tomography),and4D(time-resolved x-y-z scanning)imaging,as well as by discussing recent studies on the emerging fields of single-molecule and single-nanoparticle detection.The Review will end with an outlook on expected future developments in this exciting field of research.
2.Imaging Spatial Heterogeneities of Catalytic Solids at Different Length and Time Scales with Electromagnetic Radiation
Three criteria are important for proper chemical imaging of heterogeneous catalysts:spatial resolution,time resolu-tion,and chemical information density.Each characterization technique has its own advantages and disadvantages regard-ing these criteria.Table1gives an overview of the different characterization methods based on electromagnetic radiation available for the imaging of catalytic solids.Techniques that have already been applied in the field of heterogeneous catalysis are written in italics.The methods span the whole spectrum of electromagnetic radiation,from radio-(NMR microscopy)and microwaves(EPR microscopy)through infrared light(IR microscopy),visible and ultraviolet light (UV/Vis microscopy and different forms of Raman and fluorescent microscopy),to X-rays(e.g.STXM(scanning transmission X-ray microscopy)and TEDDI(tomographic energy-dispersive diffraction imaging)).A distinction must be made between far-field(FF)methods,which make use of a lens positioned at least a wavelength away from the object of interest,and near-field(NF)methods.Examples of the latter are scanning near-field infrared microscopy(SNIM)and scanning near-field optical microscopy(SNOM),which over-come the diffraction limits of light.All techniques rely on the absorption,scattering,fluorescence,or diffraction of light, and their abbreviations are explained in the footnotes of Table1.To give an impression of their strengths for chemical imaging,Table1also includes the currently achieved spatial resolution in the x,y,and z directions.However,spatial resolution in microscopy is almost always limited by sensi-tivity,and the ultimate resolutions reported have often been obtained for unique samples that give very large signal-to-noise(S/N)ratios.In other words,for most routine samples the practical spatial resolution is somewhat lower than indicated in Table1.
Illustrative examples on the use of space-and time-resolved in situ spectroscopy are highlighted below.These case studies will cover reactions catalyzed by acids and bases as well as by redox,electrochemical,and photochemical processes involving heterogeneous catalysts applied in the gas or liquid phase.Note that this Review is not meant to be exhaustive but merely intends to give a general overview of the exciting developments we have seen especially in the last five years.Furthermore,different high-throughput screening techniques,which often use space-resolved methods,will not
Spatial Heterogeneities in Catalysis Angewandte
Chemie
4913
Angew.Chem.Int.Ed.2009,48,4910–4943 2009Wiley-VCH Verlag GmbH&Co.KGaA,Weinheim d5bbf012b90d6c85ec3ac6cb
be covered;reference is made to articles based on IR and fluorescence microscopy.[16]
2.1.Spatial Heterogeneities at the Level of a Catalytic Reactor 2.1.1.Visualizing the Spatial Variation of Esterification,
Etherification,and Hydrogenation Reactions within Fixed-and Trickle-Bed Reactors with Magnetic Resonance Imaging Yuen et al.studied the spatial variation of a chemical conversion process within a fixed-bed reactor making use of in situ MRI.[17]To this end,they studied the liquid-phase esterification reaction of methanol and acetic acid as cata-lyzed by an Amberlyst ion-exchange resin.This liquid-phase reaction can be monitored in a reactor by following the 1H chemical shift of the hydroxy resonance associated with the fluid in the interparticle space of the catalytic bed.In this manner,it is possible to measure the extent of reaction non-invasively.Figure 3a shows a 2D slice through a 1H 3D MRI image of the fixed-bed catalyst particles (black).Three slice sections 4.5mm apart were selected,and volume-selective 1
H NMR spectra were recorded and evaluated for each of these slices.When the experiment was performed under flowing conditions,distinct spatial variations occurred within these three slice sections.These variations are evidenced by the volume-selective spectroscopy results shown in Figure 3b for slice III,in which ten volumes were selected and inves-tigated.It can be seen in Figure 3c that the peak at around 7ppm in the NMR spectrum,which arises from the 1H resonance associated with the OH group of the liquid in the interparticle space,displays different chemical shifts.This finding implies significant variation in the extent of catalytic conversion within slice III.More specifically,the fractional variation in the conversion levels reached a value of 22%.The authors applied the same approach for slices I and II in
the catalytic reactor,leading to the results summarized in Figure 3d–f.It was found that the conversion increases in the direction of the reactant flow,as expected,but that significant heterogeneity in conversion exists within each transverse section through the catalyst bed.
Koptyug et al.employed magnetic resonance spectro-scopic imaging to study the behavior of a gas–liquid–solid model catalytic reactor operating at elevated temperatures with Pd/Al 2O 3as catalyst material and the hydrogenation of a -methylstyrene (AMS)to cumene as probe reaction.[18]The experimental setup for these MRI experiments is illustrated in Figure 4a.The model reactor,which can be placed directly inside the MRI probe,consists of a system to supply H 2and liquid AMS.The research group performed a 3D MRI experiment with two spatial and one spectral coordinate.Such an experiment yields a 2D map of the liquid distribution within an axial slice through the bed,with an NMR spectrum for each image pixel acquired in the same experimental run.A 2D subset of the entire 3D dataset is shown in Figure 4b.It has one spatial (vertical)and one spectral coordinate (horizontal).The integral projection of this dataset on the vertical axis (Figure 4c)reflects the distribution of the liquid phase along the axial bar shown in Figure 4b,while any horizontal cross-section is an NMR spectrum of the liquid at the corresponding location within that bar (e.g.Figure 4d–f).For reference,the 1H NMR spectra of pure liquid AMS and cumene are included in Figure 4g,h.Despite substantial line broadening in the spectra of liquids permeating porous solids,the two spectra are still distinctly different.The essential features are 1)the presence of the broad peak at about 5.2ppm in Figure 4g and its absence in Figure 4h,2)the different separation of the outermost peaks in the spectra,and 3)the inverted ratio of their relative intensities.These spectral differences can clearly be observed in Figure 4d–f,thus indicating that AMS predominates in the upper part of the catalytic reactor;almost pure cumene is present near the
Table 1:Overview of the different characterization methods based on electromagnetic radiation available for the imaging of catalytic solids;those indicated in italics have already been used in the field.
Light source X-rays UV/Vis [b]
Infrared light
Microwaves Radiowaves Methods
X-ray micro-scopy Raman microscopy
Fluorescence microscopy
UV/Vis microscopy IR micro-scopy ESR micro-scopy NMR
microscopy currently reported far-field lateral resolution
15nm
500nm–3m m 200–300nm
1–3m m 3–5m m 3m m 3m m extension to the nanoscale possible
yes,e.g.
STXM
[a]
not yet reported yes,e.g.STED,[d]FPALM,[e]STORM [f]
not yet reported not yet reported under devel-opment
not yet reported [i]
currently reported near-field lateral resolution and related technique
under devel-opment TERS [c]
(15nm)SNOM [g](30nm)
not yet reported
SNIM [h](30nm)potential for 3D imaging and axial resolution
yes,10nm
confocal Raman (500nm)
confocal fluorescence spectros-copy (500nm);STED (20nm)STORM (30nm)
not yet reported yes
yes,3m m yes,3m m
[a]Scanning transmission X-ray microscopy.[b]A promising method to circumvent the resolution barrier of conventional diffraction is to make use of superlenses,which may bring the spatial resolution towards 10nm;see reference [110].[c]Tip-enhanced Raman spectroscopy.[d]Stimulated emission depletion microscopy.[e]Fluorescence photoactivated localization microscopy.[f]Stochastic optical reconstruction microscopy.[g]Scanning near-field optical microscopy.[h]Scanning near-field infrared microscopy.[i]After submission of this manuscript,a report appeared revealing the use of magnetic resonance imaging (MRI)with a resolution of less than 10nm on a Tobacco Mosaic virus particle;see reference [111].
B.M.Weckhuysen
Reviews
d5bbf012b90d6c85ec3ac6cb
2009Wiley-VCH Verlag GmbH &Co.KGaA,Weinheim
Angew.Chem.Int.Ed.2009,48,4910–4943
Figure 3.Illustration of the use of in situ MRI to observe in a fixed-bed reactor spatial gradients of the liquid-phase esterification reaction of methanol and acetic acid over an Amberlyst-15ion-exchange resin:a)A 2D slice through a 1H 3D RARE MRI image (RARE =rapid acquisition relaxation enhanced)of the fixed-bed reactor filled with catalyst particles,which appear black.Three slice sections for which volume-selective 1H NMR spectra were recorded are indicated along with the direction of the flow of reactants.b)The location of 10selected volumes within slice III.c)The volume-selective 1H NMR spectra for the different selected volumes in (b).d–f)Measurement of catalytic conversion within slices I,II,and III through the same bed.Reproduced from reference [17],copyright Elsevier Science B.V.,
2002.
Figure 4.a)Schematic representation of the fixed-bed reactor for measuring in situ MRI for the hydrogenation of a -methylstyrene (AMS)to cumene over a Pd/Al 2O 3catalyst.The catalyst material is placed in a glass cell.Liquid reactant (AMS)is supplied through a capillary to the top of the reactor,while a stream of hydrogen is supplied to the reactor and the temperature of the reactor volume is controlled by passing warm air through the double-walled glass cylinder surrounding the reaction volume.A thermocouple (T)is implanted into the lower part of the reactor through the supporting mesh.b)A mixed spatial–spectral 1H 2D MRI map,which corresponds to the axial bar marked in (a).c)The distribution of the liquid phase along the axial bar obtained as an integral projection of (b)on its vertical axis.d–f)1H NMR spectra of the liquid phase at different heights along the bar obtained as horizontal cross-sections of the map in (b).The location of these cross-sections are indicated by the horizontal lines in (b)and (c).The two vertical dotted lines are drawn to show the differences in the relative positions of the outermost peaks in the spectra.g,h)1H NMR spectra of AMS and cumene,respectively,obtained experimentally in solution (lower traces);the upper traces are obtained by numerically broadening the inpidual NMR lines in the solution spectra from a few Hz to 300Hz to simulate the influence of the porous matrix on the spectral line width.Reproduced from reference [18],copyright Elsevier Science B.V.,2004.
Spatial Heterogeneities in Catalysis Angewandte Chemie 4915Angew.Chem.Int.Ed.2009,48,4910–4943 2009Wiley-VCH Verlag GmbH &Co.KGaA,Weinheim d5bbf012b90d6c85ec3ac6cb
outlet of the catalytic reactor,while a mixture of the
two liquids is observed in the middle.In other words,
an increase of the product/reactant ratio from top to
bottom of the catalytic reactor could be observed by
MRI.
Two approaches are typically used when studying
hydrocarbon reactions with in situ MRI:either the
1H or the 13C nucleus can be monitored.As illus-
trated by the two examples above,whenever possible
the observation of the 1H nucleus is the method of
choice owing to its 99.9%natural abundance and the
related high MRI sensitivity.However,the disad-
vantage of 1H MRI is that the 1H nucleus has a
relatively narrow chemical-shift range.Furthermore,
there is 1)a large number of 1H resonances present in
a typical spectrum and 2)broadening of these
resonances as a result of decreased spin–spin relax-
ation times arising from the large solid–liquid inter-
face within a given sample.These detractors make
the spectral assignment and the quantification of
peak areas rather difficult,leading to complications
in the use of 1H MRI for studying catalytic reactions.
For observation of the 13C nucleus,spectral assign-
ment is easier,because 13C has a much wider
chemical-shift range than 1H,thereby reducing the
number of overlapping peaks.Unfortunately,the
natural abundance of 13C is only 1.07%,and its MRI
sensitivity is much lower than that of 1H.Molecules of interest could be enriched with 13C,but such methods are very expensive for in situ MRI experi-ments under flow conditions.To circumvent this problem,Akpa et al.devel-oped an MRI method based on the natural abun-dance of 13C,referred as 13C distortionless enhance-ment by polarization transfer (DEPT)MRI.[19]This approach has been used to monitor the competitive etherification and hydration reactions of 2-methyl-2-butene (2M2B)over an ion-exchange resin in a fixed-
bed reactor.The etherification and hydration reac-
tion products are tert -amyl methyl ether (TAME)
and tert -amyl alcohol (TAOH).Figure 5a–c shows how the double phase-encoded 13C DEPT-MRI pulse sequence ach-ieves both spatial and spectral resolution within the reactor.
Figure 5a illustrates a 1H 2D spin-echo image through the
reactor,which is overlaid with a grid showing the location of
the two orthogonal phase-encoded planes (z and x ).The
intersection of the white lines marks the center of each
volume from which the data have been taken.The corre-
sponding real-space volume elements are given in Figure 5b.
The inpidual volume elements have been separated but
actually represent a continuous array,leading to the corre-
sponding high-quality 13C NMR spectra shown in Figure 5c.It is important to note that the decreasing signal intensity
towards the walls of the bed arises from the smaller volumes from which the data are sampled.Figure 5d shows the spatially resolved 13C DEPT-NMR spectra recorded for the competitive etherification and hydration reactions of 2M2B to TAME and TAOH.The spectra were obtained for the different heights within the catalytic reactor as indicated in Figure 5a.The average conversion and selectivity from these
spectra acquired from columns 1–5at each axial location are
summarized in Figure 5e.Over a 1.5cm height of the catalytic bed,it was found that the conversion increased by about 30%,while the selectivity lies in the range of 75–80%.At any given axial position,both conversion and selectivity also vary across the transverse plane.In particular,it was found that the relatively low conversions in columns 1and 5are consistent with the faster flow rates and hence with reduced feed–catalyst contact time observed near the reactor wall.Making use of the same spatially resolved 13C DEPT-MRI method,Sederman et al.successfully investigated 1-octene
hydrogenation over a 1wt %Pd/Al 2O 3catalyst in a trickle-bed reactor.[20]Figure 6a,b shows 2D maps of 13C DEPT-MRI spectra spatially resolved along the length of the catalytic
reactor.Any horizontal cut through the 2D maps recovers a
13C 1D DEPT-NMR spectrum,as shown in Figure 6d,e.The
two solid horizontal white lines indicate the limits of the catalyst packing within the bed.Above and below the
white Figure 5.Schematic illustration of how the double phase-encoded 13C DEPT-MRI pulse sequence achieves both spatial and spectral resolution within a catalytic reactor for the competitive etherification and hydration reactions of 2-methyl-2-butene (2M2B)over an Amberlyst-15ion-exchange resin.a)A 2D 1H spin–echo
image taken through the reactor and overlaid with a grid showing the location of the two orthogonal phase-encoded planes.b)The corresponding real-space volume elements.c)The 13C NMR spectra associated with these volume elements.d)Spatially resolved 13C DEPT-MRI spectra recorded for the conver-sion of 2M2B to tert -amyl methyl ether (TAME)and tert -amyl alcohol (TAOH).The spectra are recorded at different heights within the bed.e)Conversion (*)and selectivity to TAME (&)as a function of the axial position in the catalytic
bed.Reproduced from reference [19],copyright Royal Society of Chemistry,2005.B.M.Weckhuysen
Reviews d5bbf012b90d6c85ec3ac6cb 2009Wiley-VCH Verlag GmbH &Co.KGaA,Weinheim Angew.Chem.Int.Ed.2009,48,4910–4943
lines,the reactor is packed with pure alumina support.Since the reactor was operated under H 2-poor conditions,signifi-cant conversion into the product octane was neither expected nor observed.Figure 6c shows a 1H 2D image of liquid distribution within the catalyst bed.1H signals from all liquid species present were recorded,and higher liquid content corresponds to lighter color.Qualitative interpretation of the data confirms that above the Pd/Al 2O 3catalyst material,only 1-octene exists within the bed,as identified by peaks occurring at around d =114and 139ppm.As soon as the 1-octene feed reaches the catalyst material,additional peaks in the spectrum are observed at around d =124and 131ppm,indicating the formation of predominantly 2-octene isomers.The total integrated intensity of the spectral features in the olefinic region decreases,thus confirming that some hydro-genation to octane has occurred along the length of the bed.More detailed analysis of the inpidual spectra shows 1)a loss of spectral intensity at around d =34ppm,consistent with loss of 1-octene,2)the appearance of a peak at d =18ppm confirming the formation of trans -2-octene,and 3)that relatively little 3-and 4-octenes are formed,as no spectral intensity is detected at around d =129ppm.A final example of the application of in situ MRI to study catalytic reactions in reactors involves the use of para-hydrogen (para-H 2).Gas-phase MRI suffers from low sensi-tivity,which makes it difficult to image void spaces in porous materials.This limitation has led to the use of hyperpolarized gases,such as 129Xe,3He,and 83Kr,but hyperpolarized noble gases and polarization instruments are generally expensive.
Another method for producing hyperpolarized fluids is by
using para-H 2,which has zero total nuclear angular momen-
tum (I =0)and is therefore not detectable in an NMR
experiment.This para-H 2is cheap,as it can be easily obtained
by passing hydrogen gas over an iron-containing catalyst at
the temperature of liquid N 2(à1968C)Bouchard et d5bbf012b90d6c85ec3ac6cbed
the signal enhancement by para-H 2to image a catalytic gas-
phase reaction.More specifically,they investigated the
hydrogenation of propene to propane by a silica-supported
Wilkinson catalyst ([RhCl(PPh 3)2PPh 2(CH 2)2]-SiO 2).[21,22]The
protons derived from para-H 2in the formed propane preserve
their nuclear spin singlet state.The reactant–product mixture
is adiabatically transferred to the high field of the NMR
magnet,leading to specific population differences according
to an experimental scheme referred to as ALTADENA
(adiabatic longitudinal transport and dissociation engenders
net alignment).The resulting NMR signal is enhanced
compared to what is achievable with thermal population of
spin states.It was found that a strong enhancement could be observed using para-H 2when comparing the 2D images of a propene gas-phase flow in a porous medium from the unpolarized propene and the ALTADENA-polarized pro-pane peaks.
2.1.2.Probing Rh/Al 2O 3and Rh/Pt/Al 2O 3Catalysts During the Partial Oxidation of Methane with Spatially Resolved X-ray Absorption Spectroscopy
The catalytic partial oxidation of methane over supported
noble metal particles such as rhodium and platinum is
regarded as one of the most promising processes for the production of hydrogen.Grunwaldt et al.observed a strong spatial dependence of the Rh oxidation state in a micro-reactor filled with Rh/Al 2O 3catalyst material during the partial oxidation of methane to CO,H 2,CO 2,and H 2O.2D mapping of Rh species in the catalytic reactor was possible using XANES spectroscopy (XANES =X-ray absorption near-edge structure)with a CCD X-ray camera in a reaction cell fitted with a gas supply and coupled to online mass spectrometry.[23]Figure 7a illustrates the experimental approach.The method was used to determine the distribution of oxidized and reduced Rh species as a function of position in the reactor,reaction time,reaction temperature,and space velocity.Figure 7b–g depicts the 2D X-ray transmission images of the catalyst taken at different energies.At each energy,metal particles can be identified by their specific X-ray absorption.Below the energy of the Rh K edge (23220eV ,Figure 7b),the X-ray absorption is much smaller than above the edge (23230,23235,and 23240eV ,Figure 7d–f).Moreover,the absorption is similar over the whole catalyst bed below the Rh K edge,whereas above the Rh K edge,in particular at 23235and 23240eV ,the X-ray absorption is
higher on the left side of the reactor,near the gas inlet.The higher X-ray absorption (corresponding to the brighter spots on the left)can be explained by the presence of more oxidized Rh on the left side,as oxidized Rh species are much stronger white-light absorbers.For each position in the reactor area,the X-ray absorption spectrum is reconstructed by
compar-Figure 6.2D map of 13C DEPT-MRI spectra recorded along the length of a trickle reactor bed for 1-octene isomerization and hydrogenation over a 1wt %Pd/Al 2O 3catalyst.Separate acquisitions were made for the a)olefinic and b)aliphatic regions of the 13C DEPT-MRI spectra.c)2D 1H MRI image of the spatial distribution of liquid within the
catalyst bed.Higher liquid contents are identified by lighter colors.The white horizontal lines indicate the limits of the catalyst packing,
separating the catalyst from the pure Al 2O 3.d,e)Corresponding 13C DEPT-MRI spectra measured at the interface between the 1wt %Pd/Al 2O 3catalyst and Al 2O 3corresponding to the 2D maps in (a)and (b),respectively.Reproduced from reference [20],copyright Springer,2005.
Spatial Heterogeneities in Catalysis Angewandte Chemie
4917Angew.Chem.Int.Ed.2009,48,4910–4943 2009Wiley-VCH Verlag GmbH &Co.KGaA,Weinheim d5bbf012b90d6c85ec3ac6cb
ison with the spectra of different Rh species and fitting by
least-squares methods.In this way,it has been possible to
create 2D maps of the Rh oxidation-state distribution in the catalytic reactor.An example of such a 2D map is shown in Figure 7h,i.On the right side,mainly Rh 0is found (Fig-ure 7h),while on the left side Rh 3+is detected (Figure 7i).
There is a sharp (ca.100–200m m)cone-shaped gradient of reduced Rh species.In subsequent studies,Hannemann et al.and Grunwaldt et al.showed that the position of the Rh 3+/Rh 0front is a function of the space velocity and the reaction temper-
ature.[24,25]It was found that the Rh 3+/Rh 0front moved
towards the reactor outlet (overall more Rh 3+in the reactor)
with increasing space velocity.As expected,these changes in
space velocity were reflected in the catalytic performance,
and the yield of H 2and CO decreased.Alternatively,an
increase in temperature shifts the Rh 3+/Rh 0front towards the
reactor inlet (overall less Rh 3+in the reactor).These spatial
reactor gradients can be related to the reaction mechanism.
Below the ignition temperature of the partial methane
oxidation reaction,Rh is mainly in its oxidized state.Above
the ignition temperature,the amount of H 2and CO produced
is correlated to the position of the Rh 3+/Rh 0front in the
reactor.The front shifts towards the reactor inlet when the
selectivity for H 2and CO increases.Both a catalytic
combustion and reforming mechanism and a direct particle oxidation mechanism to H 2O and CO 2have been reported.As long as Rh remains in the oxidized state,methane is totally
combusted.The appearance of H 2and CO in the gas outlet
coincides with the formation of Rh 0species in
the downstream part of the reactor,which
promotes the catalytic combustion and reform-
ing.
2.1.
3.Imaging Pt–Ba/CeO 2NO x Storage and
Reduction,Cr/Al 2O 3Alkane
Dehydrogenation,and Pt-Based Electro-
catalysts with Infrared,Raman,and
UV/Vis Spectroscopy
Urakawa et al.investigated a Pt–Ba/CeO 2
NO x storage–reduction (NSR)catalyst by a
combination of diffuse reflectance infrared
Fourier transform (DRIFT)and Raman spec-
troscopy.[26]The combined approach is based
on a switch between DRIFT and Raman
spectroscopy within a single setup.The plug
flow cell design,schematically illustrated in
Figure 8a,allows fast exchange of gaseous
atmosphere between lean (oxidative atmos-
phere)and rich (reductive atmosphere)peri-ods.The spectral detection perpendicular to the axial direction of the catalyst bed allows gradient profiling and identification of chem-ical species along the bed.During the lean periods,NO is oxidized to NO 2over platinum and stored on barium oxide in the form of
nitrates,whereas during rich periods the stored
NO x is released and reduced to N 2over
platinum;concomitantly the barium oxide is regenerated for NO x storage.Figure 8b shows the NO x concentrations during an NSR cycle.During the first 30s of the lean period,nearly complete storage of NO x was observed.Afterwards the
storage rate dropped but still showed approximately 90%
storage of the incoming gaseous NO x .Very little NO 2was observed in the off-gas during the lean period,indicating that all NO 2formed over the platinum sites was captured by the
barium oxide or CeO 2surface.After switching to rich
conditions,the NO concentration decreased rapidly.The 2D
maps of the DRIFT and Raman spectra during an NSR cycle at three reactor locations are given in Figure 8c–e and Figure 8f–h,respectively.Remarkable spectral differences were observed along the catalyst bed.The formation of nitrites and nitrates was significantly delayed and the band intensities decreased considerably on moving from the front to the back position.In the DRIFT spectra (Figure 8c–e),the most striking difference during the lean period,besides the band intensities,is the formation of nitrites.These species were observed immediately at the front position but were delayed by 70and 120s at the middle and back positions,respectively.On the other hand,nitrates were formed from the beginning of the lean period,independent of the bed position.The temporal profiles of surface nitrate bands at 1028and 1570cm à1were similar to that at 1260cm à1.The chemical changes that occur during the NSR cycle are likely accompanied by gradual structural reordering,that is,changes
in the crystallinity of the solid during nitrate penetration from the surface into the bulk of the barium oxide.
Furthermore,Figure 7.a)Side view of the experimental setup for spatially resolved X-ray absorption spectrosco-py of a catalytic reactor for measuring a Rh/Al 2O 3catalyst during the partial oxidation of methane.
The full-field imaging makes use of a position-sensitive detector and a conventional step-scanning monochromator.b–g)Flat-and dark-field-corrected 2D X-ray transmission images of the Rh/Al 2O 3catalyst inside the spectroscopic cell at different energies at 2838C.h,i)2D plots of the amount of reduced (h)and oxidized (i)Rh species obtained from the data shown in (b)–(g).Reproduced from reference [23],copyright American Chemical Society,2006. B.M.Weckhuysen
Reviews d5bbf012b90d6c85ec3ac6cb 2009Wiley-VCH Verlag GmbH &Co.KGaA,Weinheim Angew.Chem.Int.Ed.2009,48,4910–4943
during the rich period,a remarkable difference in the reduction behavior of nitrites and nitrates was detected.Nitrites were first reduced or desorbed from the surface and then the reduction of nitrates followed,as clearly indicated by a significant delay of the corresponding signals at the middle and back positions of the catalytic reactor.The large amount of NO released from the catalyst at the beginning of the rich period is likely related to the disappearance of surface nitrites,thus suggesting decomposition of the nitrites and consequent release of NO into the gas phase.Moreover,the Raman spectra shown in Figure 8f–h clearly indicate the difference in the amount of formed bulk Ba(NO 3)2.At the front position,the amount increased drastically.However,the increase was considerably less prominent towards the back position.A similar tendency is also evident in the DRIFT spectra,which show much less intense surface nitrite and nitrate bands towards the back position.Remarkably,90%of the NO x remained stored at the end of the lean period,but the majority of the NO x was stored at the front of the catalyst bed (by barium oxide).Nijhuis et al.investigated a Cr/Al 2O 3propane dehydro-genation catalyst during successive dehydrogenation–regen-eration cycles using a combination of UV/Vis diffuse reflec-tance spectroscopy and Raman spectroscopy.[27]The exper-imental setup is shown in Figure 9a.A reactor tube with optical-grade quartz windows was placed vertically in the center of a long tubular oven block that has a horizontal hole directed at the catalyst bed for 1D Raman measurements on one side and a vertical slit in the furnace for 1D UV/Vis measurements at different bed heights on the opposite side.Figure 9b shows the catalytic performance during a 4h dehydrogenation cycle.There is a clear trend in the catalytic conversion over time.The conversion increases during the first 2h and then slowly decreases.The high initial propane conversion is caused by the combustion of some propane as it reduces the catalyst.This effect also explains the lower initial selectivity.Except for water and carbon dioxide from propane combustion,the only coproducts that are observed in a significant amount are ethene and methane.The remainder of the 20%unselectively converted propane must therefore end up as coke.More direct evidence on the amount and type of coke formation could be obtained with UV/Vis and Raman spectroscopy in the reactor bed (Figure 9c–e).Figure 9c shows the UV/Vis/NIR absorbance measured at 1000nm,where no specific absorption bands are present,at four different bed heights.This absorbance was used to monitor the darkening of the sample during propane dehydrogen-ation.For Raman spectroscopy,the intensities of the two main coke bands at 1577and 1320cm à1in the middle of the reactor bed are shown (Figure 9d).The different coke formation rates at the different bed heights in Figure 9c clearly show that coke is primarily produced at the end of the reactor bed,indicating that it is formed primarily in a secondary reaction from the product propene.Figure 9d shows that initially the coke band at 1320cm à1is more
intense.As the experiment proceeds,this band becomes less intense compared to that at 1577cm à1.This relative shift is consistent with the gradual growth of a graphitic-type coke layer on the catalyst over d5bbf012b90d6c85ec3ac6cbbining the information from Figure 9b,c,it is apparent that during the first 2h of dehydrogenation coke forms slowly on the catalyst,while at the same time the catalytic activity increases.Considering that the catalyst was already reduced and that the catalyst is not reduced further than Cr 3+,the most probable explanation for the activity increase is that coke formation is beneficial for catalytic activity.After 2h of reaction,the visible amount of coke on the catalyst increases,while at the same time the conversion begins to drop.It should be noted that although after 2h the rate at which the UV/Vis absorbance of the sample increases becomes higher,this does not
necessarily Figure 8.a)Schematic illustration of the in situ IR/Raman spectroscopy reaction cell for space-and time-resolved experiments.b)NO x
concentration as a function of time for lean and rich reaction conditions.c–e)2D plots of the time-resolved DRIFT spectra during NSR at the front,middle,and back of the catalyst bed.f–h)2D plots of the time-resolved Raman spectra during NSR at the front,middle,and back of the catalyst bed.Reproduced from reference [26].
Spatial Heterogeneities in Catalysis Angewandte Chemie 4919Angew.Chem.Int.Ed.2009,48,4910–4943 2009Wiley-VCH Verlag GmbH &Co.KGaA,Weinheim d5bbf012b90d6c85ec3ac6cb
mean that the actual coke formation rate also increases.As
the selectivity of the dehydrogenation reaction does not
change,the data imply that neither more propane nor more
propene is converted into coke.The increase in absorbance
can be best explained by the coke patches increasing in size
and starting to connect and cover the entire catalyst surface,
effectively blocking the scattering and reflection of light.At
the same time,as coke starts to cover the chromium oxide,the
catalyst becomes less accessible,and its activity drops.Since
this front of complete coke coverage of the catalyst starts at
the exit of the reactor and then moves slowly forward towards
the entrance,the overall conversion drops slowly.
A final example originates from Morschl et al.,who
imaged catalytic reactions at the Pt/electrolyte interface in an
electrochemical cell using attenuated total reflection infrared
spectroscopy (ATR-IR)in combination with a focal plane
array detector.[28]This method allows collection of in situ
microscopic images of the catalyst material and its adsorbates.
More specifically,the authors investigated the electrooxida-
tion of CO over polycrystalline platinum.Figure 10shows 2D
plots of the integrated CO peak intensities versus space for
different preset currents.Red indicates a high CO coverage,
whereas blue indicates a low one.For applied currents up to
1.3mA,the CO peak intensities exhibit only slight deviations
from those of the surface with maximum CO coverage.Thus,
this region of the electrode is still completely covered by CO.
When the applied current is further gradually increased
to Figure 9.a)Schematic illustration of the in situ UV/Vis/NIR /Raman setup for measuring space-and time-resolved spectra of a Cr/Al 2O 3propane dehydrogenation catalyst in a fixed-bed reactor.b)Selectivity and conversion during a single propane dehydrogenation cycle at 5508C.c)UV/Vis/NIR trends of catalyst absorbance at 1000nm as a function of reaction time showing the coke formation at different bed heights in the reactor bed (1=top,4=bottom;see (a)).d)Raman intensities of bands of two predominant coke species as a function of reaction time.Reproduced from reference [27],copyright Elsevier Science B.V.,
2004.
Figure 10.2D plots of the integrated CO peak intensities versus space for different preset currents for polycrystalline Pt at the Pt/electrolyte interface during the electrooxidation of CO as measured with ATR-IR spectroscopy in combination with a focal plane array detector:a)0.3,b)1.4,c)1.5,d)1.7,e)1.75,and f)1.8mA.Red colors indicate a high CO coverage;blue colors indicate low CO coverage.Reproduced from
reference [28],copyright American Chemical Society,2008.
B.M.Weckhuysen
Reviews d5bbf012b90d6c85ec3ac6cb 2009Wiley-VCH Verlag GmbH &Co.KGaA,Weinheim Angew.Chem.Int.Ed.2009,48,4910–4943
1.8mA,the probed area shows two different regions:one in which the integrated intensity is still unchanged from the completely covered surface and one in which no CO peaks are detected (the region is almost free of CO).The higher the set current,the larger the region that is practically free of adsorbed CO.These regions can be correlated to activity:an inactive,CO-covered region and an active,CO-depleted region.
2.2.Spatial Heterogeneities at the Level of Millimeter-Sized
Catalyst Bodies
2.2.1.Tuning the Formation and Disintegration of Keggin-Type
Co–Mo Complexes Inside Al 2O 3-Based Catalyst Bodies During Impregnation by a Combination of Raman and UV/Vis Microscopy By making use of a combination of Raman and UV/Vis microscopy Bergwerff et al.monitored the disintegration and formation of [H 2PMo 11CoO 40]5àcomplexes inside the pores of Al 2O 3catalyst bodies.[29]The experimental approach (Figure 11)consists of taking one or more catalyst bodies
from the synthesis vessel at different times during catalyst impregnation.This step is followed by cleavage of the catalyst bodies perpendicular to their z (long)axis.Cross-section scans yield 1D plots of spectra at different positions inside the inpidual bisected catalyst bodies.The recording of such linescans with Raman spectroscopy was initially proposed by Mang et al.[30]and fully exploited by Bergwerff et al.[31]The method was later extended by Van de Water et al.[32]and Espinosa Alonso et al.[33]for recording 1D linescans with UV/Vis and IR microscopy.
When the experimental approach in Figure 11was applied to the impregnation of an aqueous [H 2PMo 11CoO 40]5àsolu-tion onto Al 2O 3catalyst bodies,Raman and UV/Vis spectra
were obtained at different positions inside the extrudates after 15and 180min of impregnation.[29]The space-and time-resolved Raman and UV/Vis spectra are shown in Figure 12.At 15min after the start of impregnation,the presence of the
[H 2PMo 11CoO 40]5àcomplex inside the Al 2O 3pores,as evi-denced by the characteristic Raman band at 971cm à1,is observed only in the outer shell of the bodies (Figure 12b).Closer to the center of the alumina bodies,Mo is present as a [H x Mo 7O 24](6àx )àspecies,as can be concluded from the position of the main Mo àO stretching vibration at 947cm à1.However,near the core of the catalyst body,the absence of any Mo àO vibration peak indicates that no Mo 6+complexes are present.These Raman microscopy observations are in full agreement with those from UV/Vis microscopy recorded at the same points of the bisected pellet 15min after the start of impregnation (Figure 12a).Indeed,a clear shoulder is observed at 17600cm à1,and the onset of the O !Mo 6+charge-transfer absorption band is shifted into the visible region of the spectrum.This finding confirms the presence of the [H 2PMo 11CoO 40]5àcomplex in the outer shell of the support bodies after 15min.In contrast,closer to the core of the pellets all Co 2+seems to be present as [Co(H 2O)6]2+,and the O !Mo 6+charge-transfer absorption band is absent,thus confirming the Mo concentration gradient observed in the Raman microscopy measurements.At 180min after the start of impregnation,a homogeneous distribution of the [H x Mo 7O 24](6àx )àcomplex is observed throughout the catalyst bodies.The intensity of the 947cm à1Raman peak relative to that of the NO 3àinternal standard is identical for all positions inside the Al 2O 3bodies (Figure 12d).This result is in line
with
Figure d5bbf012b90d6c85ec3ac6cbyout of spatially resolved measurements on catalyst bodies.An inpidual pellet is bisected or sliced after impregnation,drying,or calcination.1D spectra are then measured with Raman,IR,or UV/Vis microscopy.The slicing is performed at specific times x during the impregnation process.Reproduced from reference
[29].
Figure 12.Space-and time-resolved UV/Vis (a,c)and Raman (b,d)spectra recorded for a bisected Al 2O 3catalyst body after 15(a,b)and 180(c,d)min of impregnation with an aqueous solution containing [H 2PMo 11CoO 40]5à.The images show the catalyst bodies,and the red dots indicate the positions at which spectra were recorded.Repro-duced from reference [29].
Spatial Heterogeneities in Catalysis
Angewandte
Chemie
4921
Angew.Chem.Int.Ed.2009,48,4910–4943
2009Wiley-VCH Verlag GmbH &Co.KGaA,Weinheim
d5bbf012b90d6c85ec3ac6cb
the observation of the O !Mo 6+charge-transfer absorption band at all positions along the cross-section (Figure 12c).A homogeneous distribution of the [Co(H 2O)6]2+ion is also obtained,as the intensity of the Co 2+d–d transition at 19300cm à1is the same for all positions along the cross-section of the catalyst bodies.
The observations described above can be rationalized as follows.Phosphate is known to react with the Al 2O 3surface in acidic environments to form a surface AlPO 4phase.In this way,phosphate is withdrawn from the solution inside the Al 2O 3pores.Hence a decreasing phosphate gradient can be expected towards the center of the catalyst body.As the impregnation solution containing [H 2PMo 11CoO 40]5àcom-plexes penetrates the alumina pellets,reaction of phosphate with the Al 2O 3surface results in a lower phosphate concen-tration in the solution inside the pores of the support body.As a consequence,[H x Mo 7O 24](6àx)àand [Co(H 2O)6]2+are formed.These species make their way toward the core of the bodies,each at its own rate.Experimental evidence for the surface reaction between Al 2O 3and phosphate was found by Lysova et al.[34]using 31P MRI to monitor the dynamics of the impregnation of an Al 2O 3catalyst body in the presence of a H 3PO d5bbf012b90d6c85ec3ac6cbparison of the 1H images of water and the 31P images of phosphate revealed the strong interaction (and therefore slow transport)of the latter with the Al 2O 3support.The 2D 31P MRI images demonstrate the distribution of phosphate in the alumina pellets (Figure 13).The images in Figure 13a,b reflect the spatial distribution of the phosphate in solution only.It is expected that phosphate adsorbed on the walls of the alumina pores does not contribute significantly to the observed images.The correlation of the spatial distribu-
tions of dissolved and adsorbed phosphate has been estab-lished as well.To do so,the impregnation process was interrupted about 100min after its initiation.The selected pellet was dried and a 2D 31P MRI image of the phosphate in the solid phase was measured.The result (Figure 13c)indicates an egg-shell distribution of phosphate.In another experiment,the impregnation process was carried out to completion.The pellet was dried and imaged 18.5h after starting the process.As can be seen from Figure 13d,the liquid-phase and solid-phase distributions of phosphate do correlate with those during impregnation (Figure 13b),thus validating the applicability of MRI for dynamic impregnation studies.This study shows that the amount of free phosphate is a crucial factor in the formation of specific Co–Mo complexes within the pores of the alumina catalyst bodies.With this knowledge in mind,it was possible to form [H 2PMo 11CoO 40]5àcomplexes inside Al 2O 3catalyst bodies by increasing the amount of free phosphate in the initial impregnation solu-tion.[29]In this manner,the reaction of free phosphate with the support results in a lower phosphate concentration inside the pores,thereby creating optimal conditions for the formation of the [H 2PMo 11CoO 40]5àcomplex.
2.2.2.Altering the Macrodistribution and Speciation of Co
Complexes within Al 2O 3-Containing Catalyst Bodies during Impregnation,Drying,and Calcination as Evidenced by a Combination of Magnetic Resonance Imaging and UV/Vis Microscopy Impregnation solutions used in industrial catalyst prepa-ration processes usually contain metal precursor salts and organic complexing agents such as citrate.As a consequence,different metal chelate complexes can be formed in the impregnation solution as a function of pH value and metal-ion concentration.Since it is known that pH value gradients may occur inside catalyst bodies after impregnation,different interactions between the metal complexes and the support surface are expected.These differences may lead to different transport rates of these compounds through the support bodies.Bergwerff et al.have shown how the transport phenomena and related macrodistribution of different types of Co 2+complexes inside Co/Al 2O 3catalyst bodies can be visualized by a combination of MRI and UV/Vis micro-scopy.[35,36]While UV/Vis microscopy provided molecular information on the Co 2+complexes after bisecting catalyst bodies at specific times during impregnation,the macro-distribution of Co 2+complexes inside the catalyst bodies can be determined by MRI.Indeed,MRI can be used indirectly to image paramagnetic species,such as Co 2+complexes,as their unpaired electrons induce a decrease in the relaxation time of the 1H d5bbf012b90d6c85ec3ac6cbing a specific pulse sequence it is possible to distinguish between perturbed and unperturbed 1H nuclei of the impregnated solution occluded in the catalyst-body pores.In other words,the proportion of paramagnetic nuclei can be monitored in space and time within a catalyst body by monitoring the quenching of the 1H MRI signal.[35–37]
The proof of principle is illustrated in Figure 14for the impregnation of an Al 2O 3catalyst body with a 0.2m Co(NO 3)2solution.Figure 14a shows a set of 2D 1H images of an Al 2O
3
Figure 13.2D 31P MRI images demonstrating the distribution of
phosphate in Al 2O 3catalyst bodies.a,b)Distribution of phosphate in the liquid phase during the impregnation of the catalyst body with an aqueous solution of H 3PO 4approximately 100min (a)and 18.5h (b)after starting the impregnation process.c,d)Distribution of phosphate in the solid phase after terminating the impregnation after approx-imately 100min (c)and 18.5h (d)and drying the catalyst bodies.Lighter shades of gray correspond to higher signal intensity.Repro-duced from reference [18],copyright Elsevier,2007.
B.M.Weckhuysen
Reviews
d5bbf012b90d6c85ec3ac6cb
2009Wiley-VCH Verlag GmbH &Co.KGaA,Weinheim
Angew.Chem.Int.Ed.2009,48,4910–4943
catalyst body at different times after impregnation,while Figure 14b shows a quantitative Co 2+-ion distribution plot derived from the 2D images.It can be concluded that the transport of Co 2+ions after pore-volume impregnation takes place in two distinct steps.In a first step,Co 2+complexes are transported into the catalyst body as they travel with the capillary flow of the water solvent.Adsorption of Co 2+complexes on the Al 2O 3surface takes place as coordinating water molecules are exchanged for support oxygen atoms,and a Co 2+concentration gradient is instantly established after impregnation.Since impregnation was carried out without excess solution,the total amount of Co 2+inside the catalyst body remained constant as a function of time after impreg-nation.Therefore,the Co 2+concentration near the outer surface of the catalyst body was initially higher than 0.2m .The concentration gradient over the catalyst body resulted in diffusion of Co 2+complexes towards the center.The Co 2+front travels into the catalyst body,and after 4h a homoge-neous distribution of the metal complexes and an average Co 2+concentration of 0.2m were observed.
In a subsequent characterization study,the influence of citrate on the mobility and chemical nature of Co 2+complexes within Al 2O 3catalyst bodies was investigated.As an example,the impregnation with an aqueous cobalt citrate solution at pH 5for a cobalt/citrate ratio of 1:1will be discussed.Figure 15a shows the 2D 1H MRI image obtained from the catalyst bodies after 15h of impregnation.A sharp ring of low 1
H NMR signal intensity near the external surface of the catalyst bodies is formed immediately after impregnation.Since the red regions of the MRI image correspond to a high concentration of Co 2+complexes (blue regions indicate low
Co 2+concentrations),the 2D MRI image clearly shows a sharp egg-shell distribution of Co 2+complexes.UV/Vis spectra recorded on the corresponding bisected catalyst body show an absorption band located at around 530nm,which is due to Co 2+d–d transitions.This band is only clearly observed in the spectrum recorded near the external surface of the catalyst body,thus confirming the sharp egg-shell profile for this catalyst body.By systematic variation of the cobalt/citrate ratio and pH value of the impregnation solution,the macrodistribution of Co 2+complexes in the impreg-nated catalyst bodies could be varied from egg shell through egg white and egg yolk to uniform.
Another illustrative characterization study illustrat-ing the power of spatially resolved methods uses UV/Vis microscopy to reveal the differences in macro-distribution and speciation of Co 2+ions within Al 2O 3catalyst bodies after impregnation,drying,and calcina-tion when Co 2+-containing aqueous and ethanol solu-tions are used for impregnation.[38]Van de Water et al.found that Co 2+ions tend to migrate more slowly towards the center of the catalyst body in an ethanol impregnation solution than in aqueous solution.UV/Vis spectra of the dried samples confirmed that there are still very few Co 2+ions in the center of the catalyst body for the ethanol-impregnated sample.More inter-
estingly,however,the spectral changes observed for this sample and the complex spectra could be explained in terms of the formation of Co–Al hydrotalcite-like compounds,which are known to be formed by Co 2+-assisted alumina dissolution.By comparison,the UV/Vis spectra of the calcined sample impregnated in aqueous solution showed strong absorption bands indicative of the formation of supported Co 3O 4nanoparticles.It could be deduced that the Co 3O 4nanoparticles are rather homogeneously
distributed
Figure 14.a)2D 1H MRI images of an Al 2O 3catalyst body at different times after impregnation with a 0.2m Co(NO 3)2solution.Blue:high 1H NMR signal intensity (low Co 2+concentration);red:low 1H NMR signal intensity (high Co 2+concentration).b)Quantitative Co 2+-ion distribution plots along the position in the catalyst body,derived from the 2D 1H MRI images.Reproduced from reference
[35].
Figure 15.a)2D 1H MRI image of an Al 2O 3catalyst body after 15h of impregnation with a 0.2m Co(NO 3)2solution containing 0.2m citrate at pH 5.Blue:high 1H NMR signal (low Co 2+concentration);red:low 1
H NMR signal (high Co 2+concentration).b)Corresponding 1D UV/Vis spectra recorded on the bisected Al 2O 3catalyst body.The locations of the 1D measurement spots are indicated in the 2D 1H MRI image.Reproduced from reference [36].
Spatial Heterogeneities in Catalysis
Angewandte
Chemie
4923
Angew.Chem.Int.Ed.2009,48,4910–4943
2009Wiley-VCH Verlag GmbH &Co.KGaA,Weinheim
d5bbf012b90d6c85ec3ac6cb
over the whole catalyst body,as no major differences between edge and center were detected.In contrast,the UV/Vis spectra of the ethanol-impregnated catalyst body after calcination were much more complex,and different regions were identified.The edge of the cross-section of the bisected catalyst body contained predominantly Co3O4,whereas in other places CoAl2O4was formed.The latter phase evolved from the calcination of the Co–Al hydrotalcite compounds in places where no oxidation of Co2+occurred during drying.
2.2.
3.Revealing the Formation of Anderson-Type Aluminum
Polymolybdate and MoO3Crystallites during the
Preparation of Mo/Al2O3Catalyst Bodies by a Combination of Raman Microscopy and Tomographic Energy-Dispersive Diffraction Imaging
Bergwerff et al.investigated the impregnation,drying, and calcination of an Al2O3catalyst body with a molybdenum species.[39]Figure16a shows a typical set of1D Raman spectra recorded on bisected19wt%Mo/Al2O3catalyst bodies after impregnation with an ammonium heptamolybdate(AHM) solution at pH6.Raman bands at895and940cmà1are observed at almost all positions inside the catalyst bodies.The presence of these two bands demonstrates the coexistence of MoO42àand Mo7O246àanions inside the Al2O3pores.Inter-estingly,the Raman spectrum recorded on the outer Al2O3 surface exhibits intense bands at around947,899,572,355, and218cmà1,indicating the presence of[Al(OH)6Mo6O18]3àanions.After drying of the catalyst material,a white layer could be seen at the edges of the catalyst body,which was also visible in the corresponding scanning electron microscopy (SEM)image of a bisected catalyst body(Figure16b).The Raman spectra of this bisected catalyst body(Figure16c) indicates that the crust on the outside of the dried catalyst bodies consisted of(NH4)3[Al(OH)6Mo6O18],as evidenced by the intense Raman bands at947,899,563,and355cmà1. Similar Raman bands can also be observed in the lighter-color ring observed by SEM.In contrast,Mo7O246àcomplexes were present in the bulk of the sample,as indicated by the940cmà1 Raman band.The[Al(OH)6Mo6O18]3àcrust is formed by ligand-promoted dissolution of the Al2O3support,which results in the complexation of Al3+ions by the oxomolybdate
complexes under acidic conditions.The high Mo concentra-tion at the outside of the catalyst bodies after impregnation allows a chain reaction to occur.Al3+ions are generated by dissolution of the Al2O3support and consumed in the formation of[Al(OH)6Mo6O18]3à.The low solubility of this compound leads to the precipitation of (NH4)3[Al(OH)6Mo6O18].The Al3+concentration in the solution is thus kept low,and increasing amounts of Al2O3 are dissolved as a layer of(NH4)3[Al(OH)6Mo6O18]is formed. Inside the pores of the Al2O3toward the inside of the catalyst body,the pH of the solution increases owing to the buffering action of the support,and MoO42àcomplexes rather than Al3+ ions are formed.This species is transformed into Mo7O246àduring drying.
The egg-shell distribution in Mo/Al2O3catalyst bodies can be confirmed with TEDDI,a technique which has been explored by Beale et al.for the spatially resolved character-ization of catalyst bodies.[40]TEDDI is a noninvasive method to probe inpidual volume elements within catalyst bodies using a hard white X-ray beam(Figure17a).The TEDDI detector signal contains both the diffraction pattern and a fluorescence peak from the defined volume element in the catalyst body,as illustrated in Figure17b for the calcination of an inpidual Mo/Al2O3catalyst body.By scanning over the whole y–z area of the catalyst body,2D maps can be obtained from the Mo K a1,2fluorescence as well as from the(311)g-Al2O3and(210)MoO3diffraction peaks.As an example, Figure17c,d shows a specific y–z Mo K a1,22D fluorescence map and a y–z MoO32D diffraction map,respectively.From the latter image it can be seen that during calcination crystalline MoO3is formed from the(NH4)3[Al(OH)6Mo6O18] complexes and that these MoO3crystallites adopt an egg-shell distribution within the catalyst body.At the top and the bottom of the sample there are some brighter parts of the
Mo Figure16.a)1D Raman spectra of a bisected Al2O3catalyst body impregnated with an aqueous solution containing1.8m ammonium heptamolybdate(AHM)at pH6after1h of impregnation.b)SEM image of a bisected Al2O3catalyst body prepared in the same manner as for(a).c)1D Raman spectra recorded at different positions of a bisected Al2O3catalyst body impregnated with an aqueous solution containing1.8m AHM at pH6but now measured after drying at 1208C.Measurement positions are similar to the points labeled1–3in (b).d)1D Raman spectra of same sample as in(c)but now measured after calcination at5008C.Measurement positions are similar to the points labeled1–3in(b).Reproduced from reference[39],copyright Elsevier Science B.V.,2006.
B.M.Weckhuysen
Reviews
d5bbf012b90d6c85ec3ac6cb 2009Wiley-VCH Verlag GmbH&Co.KGaA,Weinheim Angew.Chem.Int.Ed.2009,48,4910–4943
corona,which are due to the shorter path length for the escaping fluorescence signal from the sample.This egg-shell distribution is confirmed by the 1D Raman spectra shown in Figure 16d.The Raman spectra originating from the edges of the catalyst bodies reveal the presence of bands at around 995,819,667,378,337,290,and 242cm à1,which can be assigned to MoO 3.In other areas of the catalyst bodies amorphous MoO x was detected.2.3.Spatial Heterogeneities at the Level of Micrometer-Sized Zeolite Crystals 2.3.1.Insight into Diffusion Phenomena,Surface and Internal Transport Resistance,and Pore Architecture within Zeolites by Monitoring the Concentration of Molecules with Interference and Confocal Fluorescence Microscopy Knowledge of the transport of molecules through zeolite materials and the relationship between pore structure and reactivity at the subcrystal level are important for the further development and application of such materials as heteroge-neous catalysts.Different macroscopic techniques have been used to determine the uptake and release of guest molecules within zeolites.Unfortunately,they do not provide any information on the temporal evolution of internal concen-tration profiles.A powerful method,explored K?rger and co-workers,[41–44]to reveal space-and time-resolved details,is interference microscopy.This technique is based on the principle that the optical density of an inpidual zeolite crystallite depends on the concentration and nature of the guest molecules.As a consequence,the phase shift between two light beams,one of which passes through the zeolite crystal and the other through the surrounding atmosphere,is a measure of the average guest concentration along the direction of the light beam through the crystal.The principle
of interference microscopy is schematically depicted in
Figure 18a–c.Concentration profiles at different times can
then be obtained by analyzing the interference patterns of the
two beams.Figure 18e–g shows the application of interfer-
ence microscopy to the adsorption and desorption of meth-
anol on a ferrierite crystal (Figure 18d).[41]Almost immedi-ately after the start of the adsorption experiment,a concen-tration profile associated with the roof-like part of the
ferrierite crystal is established.During desorption of meth-
anol this part of the profile disappears rapidly.Furthermore,the concentration gradients,which indicate the direction of mass transport into the crystal,are observed exclusively in the y direction of the central part of the crystal.These observa-tions can be explained by the particular features of the channels that run along the z direction of the crystal.These channels are formed by 10-rings.As the channels that run along the y direction of the ferrierite crystal are composed of smaller 8-rings,intracrystalline mass transfer is expected to proceed much faster in the z direction.Thus,the roof-like
part Figure 17.a)Layout of the experimental procedure used for measuring time-and space-resolved fluorescence and diffraction patterns from a specific volume within a catalyst body using tomographic energy-dispersive diffraction imaging (TEDDI).b)Detector signal from a Mo/Al 2O 3catalyst body after calcination at 5008C.c)Typical y –z map of the Mo K a 1,2fluorescence signals from a Mo/Al 2O 3catalyst body after drying at 1208C.d)Typical y –z diffraction map (based on the (210)reflection of MoO 3crystals)from the Mo/Al 2O 3catalyst body after calcination at 5008C.Reproduced from reference [40].
Spatial Heterogeneities in Catalysis Angewandte Chemie 4925Angew.Chem.Int.Ed.2009,48,4910–4943 2009Wiley-VCH Verlag GmbH &Co.KGaA,Weinheim d5bbf012b90d6c85ec3ac6cb
of the crystal is occupied by methanol molecules immediately
after the start of the adsorption experiment and is emptied
immediately after the start of the desorption experiment.
Another interesting feature of the concentration profiles of
Figure 18is that there are no
concentration gradients in the z
direction in the central parallele-
piped part of the ferrierite crystal.
Apparently,the channels along
the z direction are blocked in
this part of the crystal,whereas
they are wide open in the roof-
like part of the crystal.As a
consequence,any mass transfer
in the central part of the crystal
can only occur in the y direction
along the 8-ring channels.This
pore blocking pattern means that
mass transfer in the main part of
the crystal occurs only in the y
direction,even through the
molecular mobility is much
larger in the z direction because
of the larger pore system.In other
words,direct evidence is obtained
for transport resistance on the
external surface of ferrierite crys-
tals,leading to distinct guest-mol-
ecule profiles in the inner part of
the porous solid.
The same approach was used
by Tzoulaki et al.to investigate the adsorption and desorption of isobutane in coffin-shaped MFI-zeolite-type crystals.[42]Figure 19shows transient concentration profiles observed during isobutane uptake and release.Figure 19a illustrates the distribution of the isobutane con-centration within the crystal after 10s of isobutane adsorp-tion,while Figure 19b presents profiles of the
concentration Figure 18.Principle of interference microscopy applied to the adsorption of methanol as guest molecule onto a ferrierite crystal:a)Two light beams,one passing through the crystal and one through the surrounding atmosphere.b)Interference microscope.c)Interference pattern arising from the two beams passing through media with different optical properties.d)Shape and pore structure of the ferrierite crystal.e)Concentration profiles of methanol in the z direction at y =25m m;f)2D concentration profiles of methanol in the y and z directions.g)Concentration profiles of methanol in the y direction at the center of the crystal (z =120m m)and at two locations near the edges.Reproduced from reference
[41].
Figure 19.Time-dependent concentration profiles of isobutane as a guest molecule on a silicalite-1crystal:a)2D concentration profile 10s after the onset of isobutane adsorption.b)Evolution of the concentration profile of isobutane along the x axis at z =10m m during uptake.c)Silicalite-1crystal indicating the positions z of the concentration profiles in (d).d)Evolution of the concentration profiles of isobutane along the x axis during release at different z values.Reproduced from reference [42].B.M.Weckhuysen
Reviews d5bbf012b90d6c85ec3ac6cb 2009Wiley-VCH Verlag GmbH &Co.KGaA,Weinheim Angew.Chem.Int.Ed.2009,48,4910–4943
integrals along the crystal x direction.Finally,Figure19d provides a comparison of the profiles during desorption along the x axis during release at different z values.It is clear that the isobutane uptake is essentially independent of the z position in the zeolite crystal.More importantly,in both the adsorption and desorption experiments,the boundary con-centrations are found to assume the equilibrium values instantaneously.This result is different from that obtained for ferrierite and indicates that there is no additional trans-port resistance or surface barrier on the outer crystal surface. In the case of MFI-type crystallites,the observed surface diffusion barriers are probably amorphous silica layers on the external surface of the crystals.Therefore,prior to calcination and use,the crystallites were exposed to a dilute NaOH solution.During this alkaline treatment,thin amorphous silica layers easily dissolve,yielding seemingly surface-bar-rier-free crystallites.However,the work of Kortunov et al. illustrated that surface barriers could exist in MFI-type crystals.[43]By comparing the adsorption and desorption behavior of isobutane for two different ZSM-5crystals,only one of which was etched,crystal surface defects were found to either increase or decrease the rate of adsorption and desorption.The former effect could be associated with adsorption and desorption through cracks in the crystal surface,while the latter is due to blockage or structural change of the external crystal surface,leading to surface transport barriers.Furthermore,MFI-type crystals may con-tain intracrystalline diffusion barriers as well as external surface barriers.Indeed,for isobutane adsorption and desorption experiments complemented with Monte Carlo simulations,Geier et al.have been able to demonstrate that the internal intergrowth sections of MFI-type crystals do not act as molecular highways to the outer crystal surface.[44] Instead,the measured and simulated time-dependent isobu-tane profiles provided strong evidence for the adsorption and desorption of isobutane through the outer crystal surface, while the internal surfaces serve only as mild surface barriers, retarding the transport of isobutane.
Further evidence for the compartmentalization of coffin-shaped ZSM-5crystals and their related internal surface barriers was obtained by Roeffaers et d5bbf012b90d6c85ec3ac6cbing confocal fluorescence microscopy.[45,46]The liquid-phase acid-catalyzed self-condensation of furfuryl alcohol in dioxane was used as a reporter reaction for imaging the catalytic activity of ZSM-5 crystals(Scheme1).This reaction requires not only diffusion but also the availability of Br?nsted acid sites.Two furfuryl alcohol molecules initially produce a colorless bis-(furfuryl)methyl group.This molecule easily transfers a hydride ion to a primary carbenium ion formed from another molecule of furfuryl alcohol.Visible absorption then arises from the resonance-stabilized bis(furyl)carbenium ion(com-pound A,Scheme1)or its conjugated analogue(compound B,Scheme1)formed by proton loss.Excitation with visible light leads to fluorescence of compounds A and B and their related structures.The degree of oligomerization and con-jugation may increase,thus shifting the absorption and emission wavelengths of the formed chromophores towards the d5bbf012b90d6c85ec3ac6cbing confocal fluorescence microscopy,it was possible to locate in3D the emissive reaction products formed within the zeolite crystals.Some results are shown in Figure20a–c,which clearly indicates that product formation is space-and time-dependent.For a ZSM-5crystal lying on the(010)face,the optical path runs along the(100)face,and the accumulated fluorescence on this face causes a bright rim after10min of reaction(Figure20a).The spatial resolution of confocal fluorescence microscopy is sufficiently high to monitor in detail the fluorescence intensity at any point in the ZSM-5crystal.By depth profiling through the crystal or measuring line scans at different positions parallel to the(100) plane,it is possible to detect the relative intensity of the fluorescent reaction products.Clearly,a rim of fluorescent molecules is formed in the ZSM-5crystals.After16h,the fluorescence also spreads along the long axis of the crystal into the crystal edges,as illustrated by the longitudinal scans in Figure20b.It seems that any zone in the crystal can be reached by the furfuryl alcohol molecules.Interestingly,after 50days of catalytic reaction,a reversed situation is observed, and the tip segments emit the most intense fluorescence.It has been argued that at such long reaction times the microporous zeolite catalysts tend to be deactivated by pore plugging.Clearly,the desorption of product molecules from the edge segments is slow and gives rise to extensive consecutive reactions,such as oligomerization.This result is in line with internal intergrowth interface boundaries,which function as diffusion barriers that retard fast product diffu-sion.
The hydrothermal synthesis of zeolites usually requires the use of template molecules to ensure their long-range order structure;it is possible to remove these molecules by calcination.The thermal decomposition of template mole-cules leads to the formation of light-absorbing and light-emitting species.The latter,most probably conjugated aromatic compounds formed from the decomposition of charged amines,can be visualized in space and time using confocal fluorescence microscopy.As the accessibility of the porous network in the subunits varies owing to the presence of internal transport barriers for molecular diffusion,the inpidual building blocks of large zeolite crystals can
be Scheme1.Acid-catalyzed self-condensation reaction of furfuryl alcohol.
Spatial Heterogeneities in Catalysis
Angewandte
Chemie
4927 Angew.Chem.Int.Ed.2009,48,4910–4943 2009Wiley-VCH Verlag GmbH&Co.KGaA,Weinheim d5bbf012b90d6c85ec3ac6cb
visualized by monitoring the template removal process in
time.This approach was developed by Karwacki et al.and
used to reveal the intergrowth structure and related diffusion
boundaries for the structure types
CHA (SAPO-34),AFI (SAPO-5and
CrAPO-5),and MFI (ZSM-5).[47]For
ZSM-5crystals a gradual hour-glass
pattern could be revealed by perform-
ing depth scanning through the zeolite
crystal;different subunits could also be
discerned,the number and shape of
which depended on the morphology of
the zeolite crystal.
Seebacher et d5bbf012b90d6c85ec3ac6cbed 4-(4-diethyl-
aminostyryl)-1-methylpyridinium
iodide and oxazine-1(Scheme 2)as
probe molecules for studying penetra-
tion into the porous networks of large
ZSM-5and AlPO 4-5molecular
sieves.[48]Before calcination of the
molecular sieves and after immersion
into the dye solution,no fluorescence
could be detected within the solids by
confocal fluorescence microscopy.This
result is no surprise,as the dye mole-cules are too big to enter the micropore systems of these two molecular sieves.Instead,for the calcined materials both
dyes can diffuse into the inner part of
the crystals.In all cases,a rather complex and inhomogeneous band structure of fluorescence along the crystal length could be observed,which was explained by the authors in terms of the formation of internal defects in the zeolite structure.The
same 4-(4-diethylaminostyryl)-1-methylpyridinium iodide
molecule was used by Roeffaers et al.to study a series of
large ZSM-5crystals to gain detailed insight into the inter-
growth structures of these zeolites.[49]The adsorption of this
probe molecule has been linked to the visualization of straight
pores within the ZSM-5structure,and for properly treated
crystals it is argued that there are no mesopores intruding into
the crystallites.As shown by Seebacher et al.,[48]mesopores
could host these 4-(4-diethylaminostyryl)-1-methylpyridi-
nium iodide molecules.
2.3.2.Pore Alignment,Shape Selectivity,and Reactivity Patterns
within Zeolite Crystals as Probed with a Combination of
UV/Vis,Fluorescence,and Infrared Microscopy
Kox et al.[50]and Stavitski et al.[51,52]investigated large
coffin-shaped ZSM-5crystals utilizing a combination of three
in situ microscopic techniques:UV/Vis,fluorescence,and IR.
Acid-catalyzed styrene oligomerization was chosen as a probe
reaction for assessing the Br?nsted acidity in a space-and
time-resolved manner (Scheme 3).The cationic intermediates
in the styrene oligomerization reaction,which exhibit strong
optical,vibrational,and fluorescence spectra upon photo-
excitation,can act as reporter molecules for catalytic activity.
H-ZSM-5crystals exposed to 4-methoxystyrene were heated
in the in situ cell,and microscopy images as well as optical
spectra were recorded.The crystals turn purple upon heating;
however,closer examination reveals that the edges and the
main body of the crystal exhibit different colors (Figure 21
a).
Figure 20.Confocal fluorescence imaging of the condensation of furfuryl alcohol catalyzed by an inpidual H-ZSM-5zeolite crystal after a)10min,b)16h,and c)50days of reaction,together with the correspond-ing optical transmission images (grayscale)for different cross-sections and depth profiles throughout the H-ZSM-5crystal.Reproduced from reference
[45].Scheme 2.Molecular structures of a)4-(4-diethylaminostyryl)-1-methyl-pyridinium iodide,b)oxazine-1,c)terrylene diimide,d)5-carboxyfluor-escein diacetate,e)aminophenyl fluorescein,f)fluorescein,and g)resorufin. B.M.Weckhuysen
Reviews d5bbf012b90d6c85ec3ac6cb 2009Wiley-VCH Verlag GmbH &Co.KGaA,Weinheim Angew.Chem.Int.Ed.2009,48,4910–4943
As is apparent from the optical spectra in Figure 21b,two bands at approximately 590and 635nm are present in both parts of the crystal with different intensity ratios.In accord-ance with the reaction pathway in Scheme 3,the absorption band at 590nm is due to a dimeric allylic carbocation,while the band at 635nm can be assigned to a trimeric carbocation.It is evident that different parts of the crystal vary in their catalytic behavior.To better understand the nonuniform catalytic properties of the H-ZSM-5crystal,deeper insight into its microscopic structure is required.H-ZSM-5has the MFI crystalline structure with a pore system consisting of intersecting elliptical 5.3 5.1 2straight pores and 5.6
5.3 2zigzag d5bbf012b90d6c85ec3ac6cbrge MFI crystals consist of different intergrown single-crystalline subunits,which are rotated by 908about the common crystallographic [001]axis (Fig-ure 21c,d).Further experimental evidence for this inter-growth structure could be obtained using a combination of electron back-scatter diffraction (EBSD)and focused ion-beam (FIB)milling experiments.[53]As the oligomerization reaction is likely to occur within the zeolite channels,the carbocations would be expected to be entrapped and aligned within the pores.Such an alignment may be revealed using optical absorption measurements with polarized light,as the photoactive molecules absorb light polarized parallel to their dipole moment vector.Microphotographs taken with unpo-larized and polarized light (Figure 21a,e)show that the body of the crystal (an upper surface of the smaller subunit)remains translucent when the polarization is parallel to the
crystal long axis but is strongly colored when the polarizer is
directed otherwise.This finding directly implies that in this region the carbocations are aligned with the straight channels.
At the edges of the crystal,the absorbance does not change dramatically upon rotation of the polarizer (Figure 21e),thus
indicating that the preferred molecule orientation is collinear with the incident light.The intergrowth structure suggests that at the edge regions straight pores,which accommodate the intermediate molecules,are opened directly to the outer surface,while in the main body they run parallel to the surface and are accessible through the sections of zigzag pores.On the basis of this information it can be concluded that at the crystal edges the carbocation dimer is trapped within the straight pore near the surface,thus blocking further access of monomers and suppressing the formation of higher oligomers.In the crystal body,even after the dimer has been formed in the straight channel,more monomer molecules can diffuse through the sections of the adjacent zigzag pores.
In an extension of the studies of the H-ZSM-5/4-methoxy-styrene system,the factors influencing catalytic activity and selectivity were investigated using styrene derivatives bearing different substituent groups.[51]To this end,a series of large H-ZSM-5crystals was loaded with different styrene derivatives.
After reaction,the zeolite crystals exhibited colors ranging from yellow-brown through blue to pink-purple (Figure 22a).In all cases that led to catalytic reactivity,a nonuniform coloration was observed.Two factors account for the observed differences.Firstly,very bulky heavily substituted styrene molecules are not able to diffuse into the pores of the zeolite,resulting in translucent zeolite crystals.Secondly,the stability of the initial styrene carbocation determines the reactivity within the pore channels.In the case of 4-chlorostyrene,the position of the
electron-withdrawing Scheme 3.Acid-catalyzed oligomerization of
styrene.Figure 21.a)Optical microphotograph of a H-ZSM-5crystal after addition of 4-methoxystyrene,showing the different colors of the body (blue)and the edges (purple).b)Spatially resolved optical absorption spectra measured along the long axis of the crystal after reaction at the edges (solid line)and the center (dotted line);c,d)Three-dimen-sional model of the intergrowth structure of an inpidual H-ZSM-5crystal.e)Microphotographs of H-ZSM-5exposed to 4-methoxystyrene and measured with polarized light (polarization is indicated by arrows).f)Angular dependence of the optical absorption at 590nm in the body (squares)and at the edges (triangles)of the crystal.Reproduced from reference [50].Spatial Heterogeneities in Catalysis Angewandte Chemie
4929Angew.Chem.Int.Ed.2009,48,4910–4943 2009Wiley-VCH Verlag GmbH &Co.KGaA,Weinheim d5bbf012b90d6c85ec3ac6cb
halogen atom on the aromatic ring destabilizes the carbocat-ion.In contrast,when the chlorine atom is placed at the meta -position,it destabilizes the initial styrene carbocation,and no reactivity is detected for 3-chlorostyrene.The nonuniform coloration arising from the intensity variations of different absorption bands is illustrated in Figure 22b.For all styrene molecules investigated,one absorption band (assigned to the dimeric carbocations)dominates the edge spectra,while in the center other bands at longer wavelength appear,attrib-uted to species undergoing further oligomerization.To gain more insight into the oligomerization kinetics for each of the styrene compounds,Stavitski et al.performed time-resolved experiments.[51]From these data,reaction rate constants for the different reactive styrene compounds were obtained by fitting kinetic profiles with a second-order rate equation (Figure 22c).These reaction rate constants have been ration-alized in terms of the stabilization of the initial styrene carbocation.The diffusion properties of the styrene molecules also influence the catalytic activity,as can be seen from the comparison of the reactivity of 4-methoxystyrene and 4-ethoxystyrene.The 3D volume reconstructions of the con-focal fluorescence measurements for 4-methoxystyrene and 4-ethoxystyrene (Figure 22d)show that in the former case the oligomerization reaction occurs in nearly the entire volume of the crystal,while in the latter only a thin near-surface layer of the zeolite crystal is affected.Clearly,internal surface boundaries can be observed.
UV/Vis and fluorescence microscopy methods have been very powerful in the spatially resolved visualization of catalytic activity within zeolite crystals.However,extra measures are required to unambiguously unravel the chem-ical nature of the species formed during reaction.This could be accomplished with IR microscopy using synchrotron radiation.[52]H-ZSM-5crystals were exposed to 4-fluorosty-rene;corresponding IR spectra from a region in the center of the crystal are given in Figure 23a.Two sharp features,at 1534cm à1(with a shoulder at 1521cm à1)and 1510cm à1,are visible in the spectrum.The broad bands (1600–1700and 1000–1300cm à1)are attributed to the harmonics of the zeolite framework.By comparing the IR spectrum with that of 4-fluorostyrene (Figure 23a),the band at 1510cm à1is found to coincide with that of the reactant.When the spectra are recorded in situ during the reaction (Figure 23b)at 1008C,the intensity of the 4-fluorostyrene band decreases,while the band near 1534cm à1and corresponding to oligomeric styrene species increases in intensity.As the synchrotron IR radiation is intrinsically polarized,polarization experiments can be performed.Figure 23c shows IR spectra recorded with light polarized parallel and perpendicular to the long crystal axis.The intensity of the 1534cm à1band depends upon IR
light
Figure 22.a)Optical microphotographs of the H-ZSM-5crystals after styrene oligomerization with 14different styrene derivatives (1:4-methoxy-styrene;2:4-ethoxystyrene;3:4-methylstyrene;4:4-bromostyrene;5:4-chlorostyrene;6:4-fluorostyrene;7:b -methoxystyrene;8:trans -b -methylstyrene;9:3-chlorostyrene;10:2,3,4,5,6-pentafluorostyrene;11:a -methylstyrene;12:3-trifluoromethylstyrene;13:3,4-dichlorostyrene;14:2,6-dichlorostyrene).b)Optical absorption spectra of the H-ZSM-5crystals after reaction with 4-bromostyrene (4)and 4-chlorostyrene (5)at 1008C taken at the main body (black)and edges (red)of the crystal.The gray areas indicate the excitation wavelength range used in the
fluorescence microscopy experiments (510–560nm).The green bar indicates the laser wavelength in the confocal fluorescence measurements (561nm).c)Time evolution of the optical absorption band assigned to the dimeric carbocation for 4-bromostyrene (4)and 4-chlorostyrene (5).d)Confocal microscopic images of H-ZSM-5zeolite crystals measured in the spectroscopic cell after oligomerization of 4-methoxystyrene (1)and 4-ethoxystyrene (2).Two cross-sections are shown,as indicated in the corresponding 3D model.Reproduced from reference [51].
B.M.Weckhuysen
Reviews
d5bbf012b90d6c85ec3ac6cb
2009Wiley-VCH Verlag GmbH &Co.KGaA,Weinheim
Angew.Chem.Int.Ed.2009,48,4910–4943
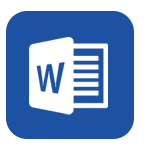





正在阅读:
Chemical Imaging of Spatial Heterogeneities in Catalytic Sol04-06
线性代数中的重要概念10-19
论债权让与中债务人保护的正当性03-31
画图解题有时也便捷06-11
办公系统工作亮点提纲合集02-23
标点符号使用中常见失误例析与训练08-30
- 1ionic liquid immobilized catalytic system for biomimetic dihydroxylation of olefins
- 2ORACLE SPATIAL常见使用错误解决办法
- 3Oracle Spatial GeoRaster 10g影像数据管理
- 4This is page 1 Printer Opaque this Empirical Bayesian Spatial Prediction Using Wavelets
- 5ORACLE SPATIAL常见使用错误解决办法
- 6This is page 1 Printer Opaque this Empirical Bayesian Spatial Prediction Using Wavelets
- 7A cosmic abundance standard chemical homogeneity of the solar neighbourhood & the ISM dust-
- 8ACHIEVING SCALABLE PARALLEL MOLECULAR DYNAMICS USING DYNAMIC SPATIAL DOMAIN DECOMPOSITION T
- 9Gold nanocluster-based fluorescence biosensor for targeted imaging in cancer cells and ratiometr - 图文
- 10Closing in on a Short-Hard Burst Progenitor Constraints from Early-Time Optical Imaging and
- exercise2
- 铅锌矿详查地质设计 - 图文
- 厨余垃圾、餐厨垃圾堆肥系统设计方案
- 陈明珠开题报告
- 化工原理精选例题
- 政府形象宣传册营销案例
- 小学一至三年级语文阅读专项练习题
- 2014.民诉 期末考试 复习题
- 巅峰智业 - 做好顶层设计对建设城市的重要意义
- (三起)冀教版三年级英语上册Unit4 Lesson24练习题及答案
- 2017年实心轮胎现状及发展趋势分析(目录)
- 基于GIS的农用地定级技术研究定稿
- 2017-2022年中国医疗保健市场调查与市场前景预测报告(目录) - 图文
- 作业
- OFDM技术仿真(MATLAB代码) - 图文
- Android工程师笔试题及答案
- 生命密码联合密码
- 空间地上权若干法律问题探究
- 江苏学业水平测试《机械基础》模拟试题
- 选课走班实施方案
- Heterogeneities
- Catalytic
- Chemical
- Imaging
- Spatial
- Sol
- 【非常考案】(通用版)2022版高考生物一轮复习分层限时跟踪练27水
- 造价工程师《工程造价计价与控制》章节知识:第四章第三节试题
- 第一节实践是人生修养的现实基础和检验标准
- 兰州七里河安宁污水处理厂TOT项目融资案例分析报告
- 工业通信业行业标准制定管理办法
- 大学生预备党员转正申请书字数5000字
- 一年级下册人与自我全册教案
- 关于保护环境的温馨提示语【可编辑版】
- 六年级科学下册试题 - 期末复习一 放大镜的使用 教科版(无答案
- 《CAXA电子图版》教学设计
- 2022年聊城大学概率论(同等学力加试)考研复试核心题库
- 历年高考试题《三角函数》整理
- 制药年产25吨氢化可的松的车间工艺设计文献综述2详解
- 公式及其适用条件总结(1)
- 数学实验操作性教学对课堂作用的探究
- 2022年吉林大学艺术学院628中外音乐史之西方音乐史简编考研冲刺
- 造口伤口专业管理小组工作方案
- 开工典礼仪式主持词
- 什么样的电子版合同有效
- 高中语文课外古诗文《史记蔡泽传》原文及翻译